New research uses CRISPR gene editing to grow new neurons in diseased brains
Scientists hope the CRISPR-based therapy could treat neurodegenerative disease
The brain is a marvel of evolution, but in some animals, it has a few limitations. Unfortunately for humans, our brains are mostly incapable of generating new neurons. This inability becomes particularly problematic when the brain is affected by neurodegenerative disorders.
To treat neurodegenerative diseases, scientists can create new neurons from stem cells. In no time, new neurons can replace lost ones, and take over their job. A glaring drawback of this method is that these cells are formed outside the body and need to be transplanted into the brain. To date, this is the preferred method to repopulate lost neurons, but it’s far from ideal.
Transferring lab grown neurons into animal brains reduces the cells' viability — their chances of integrating well into the tissue — and the efficiency with which they can restore function. So scientists at Shanghai Research Center for Brain Science and Brain-Inspired Intelligence fashioned a method to regenerate neurons inside the brain. The method is similar to how one would revive a dying plant: by nurturing it with the right conditions for it to grow new leaves.
Building up on a previous study, Haibo Zhou, a postdoctoral researcher in Hui Yang’s lab, and colleagues, set up a method to convert non neuronal brain cells called “glia” into neurons. They did this by turning down a gene called PTBP1 in glia of different parts of the mouse brain, using the gene-editing tool CRISPR. Depending on which brain region was targeted, the glia gave rise to different kinds of neurons.
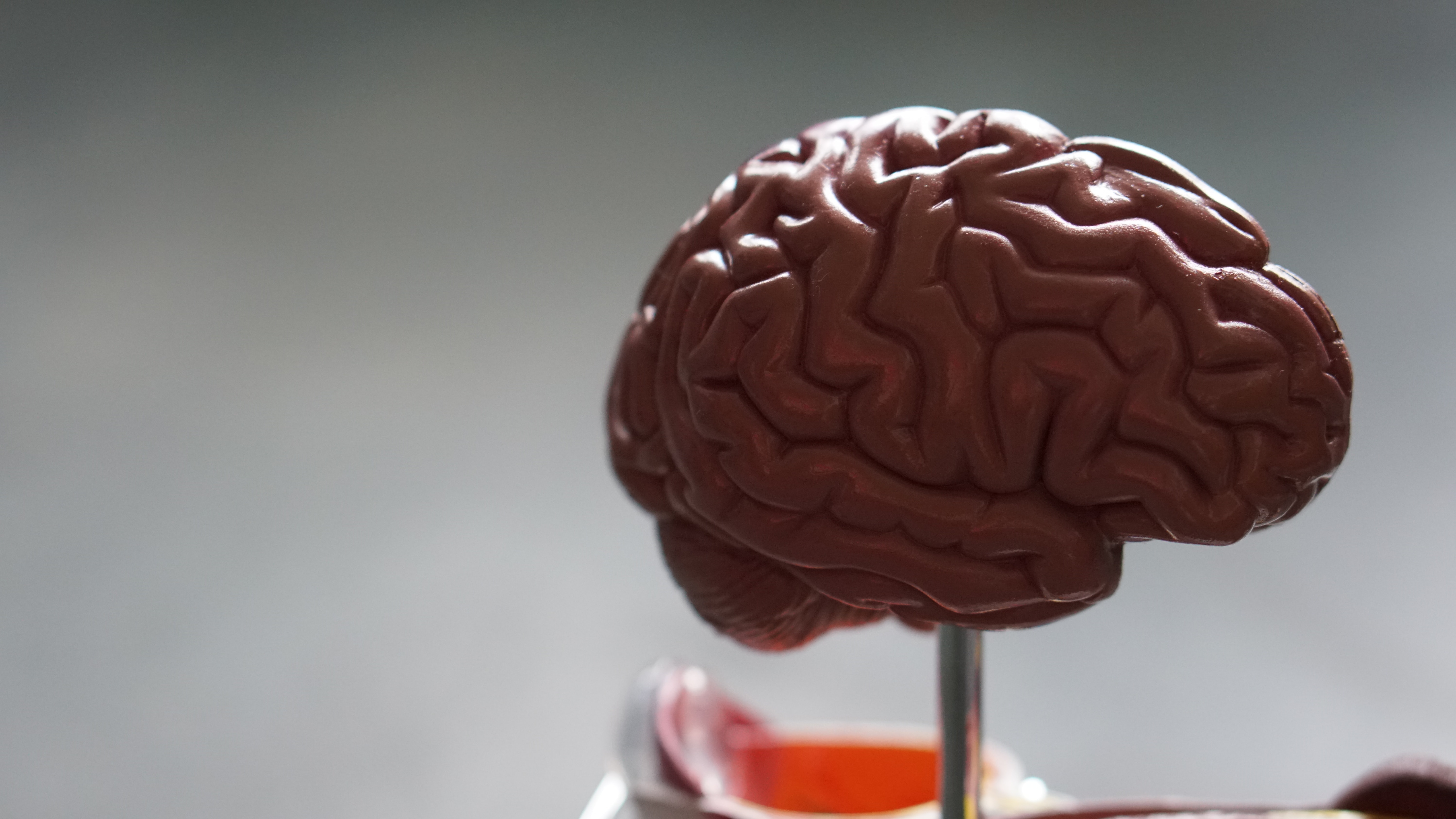
The method partially restored the normal motor behavior of the anima
Photo by Robina Weermeijer on Unsplash
Reducing PTBP1 levels presumably reverted glia to unspecified stem cells, which adopted varied neuronal identities based on which glia were targeted and the environmental signals they received. This was evident from the team’s successful attempts at restoring two different types of neurons and alleviating the symptoms associated with the loss of each.
Parkinson’s disease occurs due to loss of dopamine-producing neurons and manifests as tremors, stiffness, and loss of balance. To test their method in rejuvenating this group of neurons, the team first got rid of them using a toxic compound in mice. The authors then converted glia into dopamine-producing neurons, and the new cells showed the same activity as their original counterparts.
This rescue was not limited to just the neuron population. It also partially restored the normal motor behavior of the animal. This is a huge step forward from drug induced alleviation of symptoms because it puts forth a more permanent solution.
The team also tackled retinal diseases caused by death of retinal ganglion cells, or RGCs, which leads to permanent blindness. Turning down PTBP1 in glia of the retina transformed them into RGCs. Astoundingly, these renewed neurons not only responded to light independently, but also sent their projections to the visual cortex correctly, restoring circuit function. This led to a partial recovery of eyesight in the treated mice.
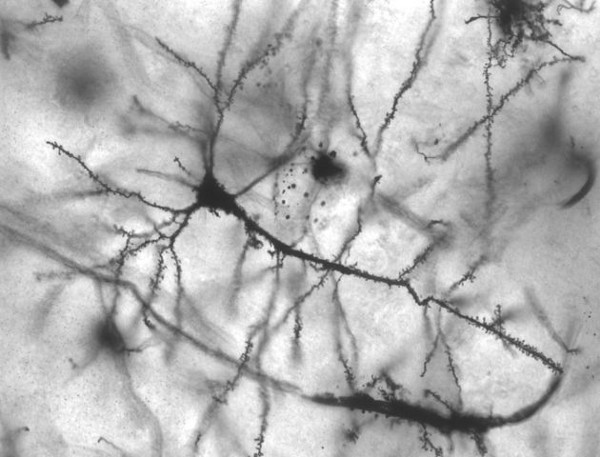
Neurons in different regions of the brain vary a lot in their shapes, activity, function, and connectivity
Image by MethoxyRoxy, reproduced under CC BY-SA 2.5
The outcomes from this study are surprising in many ways. Neurons in different regions of the brain vary a lot in their shapes, activity, function, and connectivity. Neurons in young animals acquire these characteristics because of the multitude of developmental signals they receive. These cues might or might not be present in the adult brain. How do the reprogrammed neurons pull this off in the absence of such cues?
“Maybe the signals still exist in the mice at 8 weeks old. It’ll be very interesting to identify the instructive signals underlying glia-to-neuron conversion in the future”, Yang said in an email. Looking at natural regeneration in animals like zebrafish, where a similar mechanism of glia-to-neuron transformation is observed, could give us hints to what these signals might be.
The high efficiency and flexibility of this technique are appealing. But, given the notoriety CRISPR-based gene editing has garnered in the recent years, the safety of translating the technology into other methods is an open question. How will researchers ensure the system only edits the correct gene? How would one stop the editing once neurons are regenerated?
The authors chose to reduce the levels of PTBP1 by targeting the RNA, rather than DNA. For this they chose Cas13d because it's small, easy to deliver to the cells, yet highly specific and efficient in its action. Compared to conventional tools like Cas9, it's a significant improvement.
According to Yang, CRISPR RNA editing is safer than DNA editing since expression of Cas13d is turned down once neuronal conversion is complete. Unlike DNA editing, which alters the genetic makeup of an animal permanently, RNA editing affects the expression levels of specific genes, temporarily.
“We are now working on monkey model using this approach," Yang says. "Hopefully, we could apply this approach in humans in three to four years.”
If meticulously designed experiments provide evidence for safe therapeutic use in humans, it might open doors for using this method to generate various other cells of the body as well. Treatment options for disease like diabetes and sickle cell anemia could be well within reach. But that is a story for another time.
It is exciting to see Cas13d technology being used in live animal model research, with translational intentions. I appreciated that you drew attention to this as a possible solution to the risks/impracticality of using Cas9 for gene editing in humans. It will be nice to see how this method translates into larger mammals! This article also has me wondering if there might be a method to deliver Cas13d to the glia other than the method the authors used here, given some of the challenges of targeting the central nervous system with AAV-mediated gene therapies.